Stem Cell Biotechnology 101: Stem Cells in Regenerative Medicine
Foreword
The field of stem cells is brimming with promise and intrigue. As the understanding of these remarkable foundational components of life continues to evolve, so does the capacity to harness their extraordinary potential. Stem cells have enchanted the minds of scientists, medical professionals, and curious individuals alike, presenting unparalleled opportunities to revolutionise healthcare and regenerative medicine. This series of articles aims to unravel the mysteries of stem cell differentiation, explore the mechanisms governing their self-renewal, and examine the factors that shape their destiny. Within these articles, readers may discover the immense potential of induced pluripotent stem cells (iPSCs) in reshaping personalised medicine while uncovering their awe-inspiring regenerative capabilities. Moreover, this series sheds light on the critical challenges and limitations inherently intertwined with this field, from ensuring the safety and efficacy of stem cell therapies through navigating the complex terrain of regulatory frameworks and public perception to debating ethical uncertainties.
This 101 series is divided into eight articles:
6. Stem Cell Biotechnology 101: Stem Cells in Regenerative Medicine
7. Stem Cell Biotechnology 101: Stem Cells in Disease Modelling and Cancer Research
8. Stem Cell Biotechnology 101: Ethical Considerations and Future Perspectives
Stem Cell Biotechnology 101: Stem Cells in Regenerative Medicine
The concept of regeneration has captivated the human imagination for centuries, evolving from mythological tales to tangible scientific pursuits that command credibility today. Regenerative science forms an intricate ecosystem founded on the principles of discovery, translation, and practical application, bolstered by a well-established supply chain, cumulative regulatory and clinical expertise, and heightened general awareness. With a versatile toolkit encompassing innovations like nanotherapies and artificial organs, regenerative science is poised to enhance healthcare systems. This vision thrives on the synergy of research innovation, workforce education, and the implementation of regenerative practices [Figure 1]. Regenerative technologies play a transformative role in clinical practice, providing tools to restore normal organ function while emphasising comprehensive patient care. Clinical advancements have introduced innovative therapeutics while adhering to a comprehensive assessment framework. In this burgeoning landscape, there is a growing emphasis on the holistic evaluation and incorporation of the patient into the care regimen, addressing real-world patient needs and mirroring society's overarching quest for value-based healthcare (Yamada et al., 2021).

The imperative for tissue replacement or regeneration arises in various critical scenarios, spanning tissue injuries, traumatic incidents, malignancies, and age-related or degenerative maladies. Stem cells, endowed with their unique capacity for self-renewal and differentiation, are emerging as pivotal assets to rejuvenate and restore damaged tissues and organs, thus forming the cornerstone of regenerative medicine (Mahla, 2016). This burgeoning discipline represents a novel frontier in translational research, synthesizing principles from tissue engineering and molecular biology with the overarching objective of reinstating the normal function of impaired cells, tissues, or organs (De Miguel et al., 2019). A long-term vision within regenerative medicine envisions the cultivation of tissues, organ fragments, or even complete organs within the controlled confines of a laboratory, with the eventual aim of implanting them into individuals whose bodies lack the innate capacity for self-healing. This innovative approach promises to alleviate the persistent challenges associated with organ shortages for transplantation while potentially mitigating the complications tied to graft rejection, especially when the organ can be cultivated from the patient's autologous cells, circumventing histocompatibility concerns (M. Ratajczak, 2019).
Principles of Regenerative Medicine
Regenerative medicine, often regarded as the vanguard of medical science, is a field that revolves around the intricate task of restoring the functionality of damaged or diseased tissues and organs. It comes into play when the body's innate regenerative responses are insufficient to heal severe injuries or combat chronic diseases, marking a critical juncture in healthcare innovation and patient care (Mason & Dunnill, 2008). There is a significant gap between the demand for transplantable tissues and organs, driven by ageing and illness, and the available supply. Regenerative medicine is dedicated to the task of repairing or replacing damaged tissues and organs, offering a promising solution to these pressing healthcare challenges. It represents the forefront of medical innovation, potentially improving the quality and length of patients' lives, especially those grappling with conditions that were once considered insurmountable barriers to health and well-being (Edgar et al., 2020).

Promoting healthy ageing has become a pressing concern on a global scale, especially as the elderly population deals with a high incidence of chronic diseases (Terzic & Waldman, 2011). Recent scientific breakthroughs, backed by stringent regulatory oversight, ethical considerations, and a focus on quality control, have led to the development of various regenerative biotherapy pipelines. Regardless of the specific approach, these therapies share a common thread: they harness and amplify the body's inherent regenerative capabilities to enhance its natural healing and repair mechanisms (Wells & Watt, 2018). The overarching mission of regenerative medicine is to adopt a proactive approach that revolves around prevention, protection, and promotion of well-being, aiming to extend disease-free years of life [Figure 2]. Rooted in the aspiration for patients to regain their health, this regenerative perspective offers a versatile framework applicable to a broad spectrum of diseases, thereby reshaping perceptions of future healthcare (Hargraves et al., 2018).

The leading ambitions of regenerative medicine can be classified into two approaches (Baraniak & McDevitt, 2010) [Figure 3]. The first focuses on unlocking the incredible potential of stem cells to respond effectively in emergencies, facilitating the rapid regeneration of damaged or compromised tissues. This method is an immediate application, introducing stem cells directly into the patient's body to address acute health crises, such as heart attacks or traumatic injuries. The second goal extends its emphasis to the adult stem cell compartment, aiming to modulate and optimise its performance to ensure the enduring vitality of stem cells throughout adult life. This approach encompasses a broader, more holistic strategy involving both in vitro and in vivo methodology. It is focused on long-term regenerative enhancement and often targets chronic conditions, aiming to improve the patient's quality of life while balancing the potential side effects of treatments. Recent advancements in stem cell biology and technological innovations have breathed fresh life into regenerative medicine, ushering in an array of exciting opportunities in advanced tissue engineering and the development of cutting-edge cell-based therapies. These dual pursuits underscore the profound impact that regenerative medicine is poised to have on the future of healthcare, holding the promise of not only addressing acute crises but also fortifying the human body's natural regenerative abilities in the long term (Ratajczak, 2019).
Stem Cells - The Cornerstones of Regeneration
Stem cells, praised for their extraordinary capacity to tackle diverse medical obstacles, assume a central and indispensable role in regenerative medicine. In humans, as in other sexually reproducing species, the development journey launches from a solitary, rudimentary entity—the fertilised oocyte (Ratajczak et al., 2016). This remarkable unicellular entity called the zygote possesses an innate potential since it can both multiply and specialise, orchestrating the genesis of the entire array of specialised cell types that constitute the mature human body. This extraordinary attribute of a cell is termed "totipotency" (Condic, 2014).

As demonstrated throughout this 101 series, stem cells are the fundamental building blocks of biological organisation and epitomise the exceptional amalgamation of self-renewal proficiency and the potential for differentiation into diverse cellular lineages (Zakrzewski et al., 2019). This dual functionality bestows them an indispensable role in the multifaceted processes governing organ and tissue system development and regeneration. The vitality of the adult stem cell reservoir stands as a key determinant, exerting a substantial influence on both the quality of life and the overall lifespan. Their proper functioning is essential for achieving healthy ageing (Kucia et al., 2011). Notably, the orchestration of continuous renewal of differentiated cells within adult tissues varies across organs, following a distinctly choreographed tempo of replacement (Visvader & Clevers, 2016). It is paramount to acknowledge that diverse tissues employ a range of discrete strategies to effectuate self-renewal and repair, each finely tuned to the unique exigencies of their respective microenvironments.
For approximately five decades, the impressive achievements of hematopoietic stem cells (HSCs) in hematopoietic transplants have not only been a source of inspiration but have also ignited a growing interest in the potential of adult stem cells to address a broad range of medical challenges (D’Addio et al., 2014; Hofmann et al., 2005). These efforts encompass a spectrum of conditions, including the rejuvenation of damaged myocardium following heart infarction, the recovery of neural tissue post-stroke, the restoration of spinal cord function after mechanical injuries, and the treatment of age-related macular degeneration in the retina. Moreover, adult stem cells show promise in the ongoing battle against diabetes, assisting in the healing of extensive skin burns, rejuvenating impaired liver tissue, and alleviating the effects of Parkinson's disease. These pioneering therapies primarily rely on adult tissue-derived stem cells from various locations, such as bone marrow, mobilised peripheral blood, umbilical cord blood, adipose tissue, and even myocardial biopsies (Ratajczak et al., 2016). While this represents an exciting chapter in medical science and patient care, it is indispensable to remember that the effectiveness of these treatments may vary, and their widespread adoption is an evolving process. Ongoing research and clinical trials provide valuable insights into their full potential.

Organ Transplantation: A Brief History
Organ transplantation faces significant challenges, stemming primarily from the overwhelming demand for donor organs and the quest for immunosuppression-free strategies. The historical trajectory of organ transplantation can be divided into three distinctive phases [Figure 6]. The initial phase, rooted in the early days of surgical science, marked the "surgery era." While the technical feasibility of transplantation was demonstrated during this era, progress was hindered by the absence of effective antirejection therapies until the emergence of ciclosporin, which propelled the field into the 'immunology era.' Immunosuppression revolutionised transplantation, making it a standard of care for numerous medical conditions, although it introduced its own set of side effects. Consequently, extensive research has centred on achieving immune tolerance to enable immunosuppression-free status, where recipients can accept transplants without needing immunosuppressants (Edgar et al., 2020).

More recently, the field has been transitioning into the "regenerative era," coinciding with advancements like big data utilisation, exchanged pair donation chains, and cross-blood group or incompatible donor transplants (Bozek et al., 2018; Massie et al., 2014; Vincenti et al., 2016). Notably, organ bioengineering and regeneration technologies have made remarkable strides in manufacturing organs using a patient's own cells, potentially paving the way for on-demand organ creation and immunosuppression-free transplantation. Long-term management of transplant recipients focuses on reducing morbidity and mortality, enhancing quality of life, and carefully balancing the side effects of immunosuppressive medications with the risk of graft failure. This approach to chronic disease management might be described as a "halfway technology," as it does not address the underlying disease directly and may induce life-altering adverse effects (Asch & Volpp, 2017). The advent of regenerative medicine promises to transform organ transplantation into a "full-way technology," potentially eliminating the need for ongoing medication. While the feasibility of this approach has been demonstrated through the implantation of segments of the urinary tract or upper airways, it is believed that it is only a matter of time before complex organs can be bioengineered (Orlando, Baptista, et al., 2011; Orlando, Wood, et al., 2011).
As of January 12, 2021, data from the United States Organ Procurement and Transplantation Network revealed a waiting list of 118,618 patients, with a new addition every 9 minutes despite approximately 100 daily transplants. The chasm between organ procurement and patient demand, exacerbated by the requirement for chronic immunosuppression, stresses the pressing necessity for alternatives to traditional transplantation (Edgar et al., 2020). In cases involving multiorgan diseases or anatomical abnormalities due to congenital malformations, therapeutic options remain limited, underscoring the alluring promise of regenerative approaches, which offer the building blocks needed to potentially restore physical and functional integrity in conditions once deemed insurmountable (Yamada et al., 2021).
Organ Transplantation: The Apparition of Stem Cells
The discovery of stem cells marked a historic milestone in medicine, making advancements in today's healthcare possible. Stem cell transplantation is now an important component of regenerative medicine, providing various strategies for tissue regeneration and combating diseases. These approaches encompass using a patient's own cells (autologous), cells from a donor (allogenic), or cells from an identical twin (syngeneic). In addition to their versatility, stem cells have unique properties that make them valuable in transplantation. They express a specific receptor known as the major histocompatibility complex (MHC) at relatively low levels. The role of this receptor is a fundamental aspect in evaluating the compatibility of transplanted cells with the recipient's immune system, a pivotal factor influencing the efficacy of these treatments. Whether the MHC is present or absent can profoundly influence stem cell transplantation results. Consequently, comprehending the significance of the MHC holds immense importance for healthcare providers and patients alike. On top of it, stem cells release chemical signals known as chemokines, which are key in attracting endothelial cells and immune cells. This process creates a conducive environment for tissue acceptance at the graft site, reducing the risk of rejection (Petersdorf et al., 2007).

Modern regenerative medicine heavily relies on innovative tissue engineering techniques. These methods blend cell transplantation, materials science, and microengineering to produce organoids that faithfully replicate the function of damaged tissues and organs, thus restoring their normal function. The process involves creating new tissue on biodegradable 3D scaffolds that are thoughtfully designed to support cell adhesion, replicate the mechanical properties of the target tissue, promote angiogenesis, and ensure proper tissue perfusion. Importantly, they are intended to be non-immunogenic, reducing the need for widespread immune suppression (Garzón et al., 2012; Gubareva et al., 2016). The success of regenerative treatments is closely linked to the number of stem cells transplanted into the tissue (Thompson et al., 2016). In many cases, expanding the population of transplantable stem cells ex vivo (outside the body) is necessary before transplantation. This process significantly enhances the likelihood of success. To achieve successful regenerative outcomes, transplanted stem cells must survive, multiply, differentiate into the appropriate cell types, and integrate into the host's circulatory system (Nathamgari et al., 2015).

ESCs in Regenerative Medicine
1998 marked a significant breakthrough—James Thomson successfully isolated human embryonic stem cells (hESCs) (Thomson, 1998). These extraordinary cells are pluripotent and can give rise to over 200 distinct cell types, thanks to the dynamic interplay of transcription factors like OCT4, SOX2, and NANOG. As discussed throughout this 101 series, these factors govern the pluripotent fate of hESCs, orchestrating their remarkable versatility (Hogan et al., 2015). When subjected to specific culture conditions, embryonic stem cells can differentiate into various cell types, including hepatocytes, retinal ganglion cells, chondrocytes, pancreatic progenitor cells, cone cells, cardiomyocytes, pacemaker cells, eggs, and sperm. These differentiated cells can be employed in tissue-specific regeneration and disease treatment approaches (Mahla, 2016).
Devastating conditions like spinal cord injuries (SCIs), often resulting from infections, cancer treatments, or accidents, have witnessed a glimmer of hope via the transplantation of hESCs. Paraplegic or quadriplegic SCI patients who receive these transplants experience considerable improvements in body control, balance, sensation, and limb movements. The transplanted stem cells demonstrate an ability to home in on the injury sites, contributing to the recovery process (Shroff & Gupta, 2015).

Age-related macular degeneration (ARMD) is another area where hESCs offer hope. ARMD involves the deterioration of the retinal pigment epithelium in the central retina macula, leading to vision loss. Researchers have addressed this challenge by genetically manipulating hESCs by introducing the COCO gene. This gene, typically active during embryogenesis, is artificially incorporated into the hESCs to ensure continuous expression. This sustained COCO gene expression guides the lineage commitment of hESCs into cone cells, effectively suppressing signalling pathways like TGF, BMP, and Wnt. When these specialised cone cells are transplanted into the eye, they can reverse the effects of ARMD. These cells migrate and form sheet-like structures within the host retina, potentially restoring lost vision. However, a complex challenge remains in ARMD therapeutics: establishing the missing neuronal connections among retinal ganglion cells, cones, and the retinal pigment epithelium (Zhou et al., 2015).
Cardiovascular diseases are a leading cause of human mortality worldwide, requiring urgent therapeutic interventions to restore heart function and ensure patient survival. Cardiac tissue regeneration can be accomplished through the transplantation of various cell types—cardiomyocytes, cardiovascular progenitors derived from embryonic stem cells, and bone marrow-derived mononuclear cells. However, the healing potential of the last ones falls short compared to others. Mature cardiomyocytes exhibit a high capacity for tissue healing, arrhythmia suppression, and electromechanical integration into heart functions and provide mechanical and electrical repair without the associated risk of tumorigenesis (Fernandes et al., 2015). ESC-derived liver stem cells also show significant promise. These cells can be transformed into specific hepatocytes, which are important for modifying and breaking down toxic xenobiotic drugs (Avior et al., 2015).

ESCs also hold the potential for differentiation into insulin-secreting beta-cells, offering a promising avenue for the permanent treatment of type 2 diabetes (Bruin et al., 2015). Osteoarthritis, a condition affecting millions globally, results from the degeneration of joint cartilage, leading to joint stiffness. While current therapies for arthritis alleviate symptoms, they do not encourage cartilage regeneration. For young individuals and athletes unable to undergo joint replacement, stem cell transplantation offers an alternative for healing cartilage injuries. Chondrocytes, cartilage-forming cells derived from hESCs embedded in a fibrin gel, have effectively repaired defective cartilage within 12 weeks when transplanted into focal cartilage defects in knee joints in mice (Cheng et al., 2014). ESCs also promise to regulate heart rhythm as biological pacemakers. By coaxing ESCs into inert biomaterial and propagating them under defined culture conditions, they can be transdifferentiated into sinoatrial node pacemaker cells (Vedantham, 2015).
In short, ESCs can be transdifferentiated into a wide range of cell types representing the three germ layers of the body, making them an up-and-coming source for regenerative medicine in tissue regeneration and disease therapy. Ethical concerns limit the applications of ESCs, necessitating adherence to established guidelines. Other cell types, like mesenchymal stem cells or induced pluripotent stem cells (iPSCs), can serve as alternative approaches (Mahla, 2016).
Tissue-Specific Stem Cells in Regenerative Medicine
Tissue-specific stem cells (TSPSCs) are vital to the human body's biological machinery. They play a fundamental role in maintaining the balance and proper functioning of our various tissues and organs. In contrast to ESCs, continuous cell division sets these specialised stem cells apart while retaining their unique capacity to differentiate into a limited range of cell types specific to their particular tissue. Despite their incredible potential, TSPSCs pose particular challenges due to their limited presence in the body. The relatively low population of TSPSCs within the total cell population makes their isolation and in vitro manipulation a complex and often delicate task. This limitation accentuates the need for advanced techniques and technologies to harness the therapeutic potential of these cells (Greggio et al., 2013).

Several types of tissue-specific stem cells are present in the body, each offering distinct possibilities. For example, pancreatic progenitor cells promise to treat type 2 diabetes by giving rise to insulin-producing beta-cells (Ye et al., 2016). In a different context, dental pulp stem cells, responsible for maintaining dental health, can be reprogrammed into neurons, which could have implications for dental neurogenesis (Potdar, 2015). Exciting possibilities appear in addressing cognitive issues resulting from cancer treatments. In chemotherapy-induced cognitive impairment, colloquially known as "chemobrain," transplanting neuronal stem cells derived from humans can help restore cognitive functions and reduce neuroinflammation (Acharya et al., 2015). TSPSCs located in the inner ear could restore hearing by regenerating cochlear hair cells. Intestinal tissue-specific progenitor stem cells are indispensable for the repair of damaged intestinal tissue. By carefully controlling their culture conditions, an almost unlimited supply of these stem cells may be generated, providing a priceless resource for regenerative therapies (Mizutari et al., 2013).
Limbal stem cells, situated in the basal limbal epithelium of the eye and marked by the presence of ABCB5, play a critical role in the regeneration and maintenance of corneal tissue. Functional ABCB5 is essential for these limbal stem cells' survival and functional integrity, and their deficiency leads to corneal issues. Hence, these stem cells offer a potential solution for restoring vision (Ksander et al., 2014). For conditions like Duchenne muscular dystrophy, which results in extensive muscle tissue damage, tissue engineering technologies can be employed to promote functional restoration through tissue regeneration. The method might involve encapsulating mesoangioblasts derived from either mice or humans, engineered to express placental-derived growth factor, in polyethene glycol fibrinogen hydrogel. When transplanted beneath the skin, these cells can form artificial muscles that function similarly to normal muscles (Fuoco et al., 2015). Spermatogonial stem cells demonstrate their versatility by giving rise to various cell lineages depending on the surrounding tissue's microenvironment. The transplantation of spermatogonial stem cells, combined with the mesenchyme from the prostate, skin, or uterus, can lead to the differentiation of these cells into the epithelial cells specific to the tissue of origin. This compelling research highlights the vital role of niche effects in regenerative medicine (Simon et al., 2009).

The eye is a susceptible organ, and conditions such as diabetic retinopathy can lead to progressive vision loss due to reduced vascularisation within the retina. The intravitreal injection of adipose-derived stem cells has demonstrated the ability to restore microvascular capillary beds in mice. Interestingly, cells obtained from healthy donors produce higher amounts of vasoprotective factors than those from individuals with high blood sugar levels. Still, using adipose-derived stem cells for therapeutic purposes requires further standardisation, particularly in determining the optimal cell counts for transplantation and closely monitoring the outcomes on a broader scale (Cronk et al., 2015). Another area of research involves skin-derived precursors (SKPs), which are the progenitors of dermal papilla, hair, and hair sheath. SKPs can give rise to multiple tissues originating in both the mesodermal and ectodermal layers, such as neurons, Schwann cells, adipocytes, chondrocytes, and vascular smooth muscle cells (VSMCs). Specifically, VSMCs have shown promise in wound healing and angiogenesis. When derived from human foreskin progenitor SKPs, these VSMCs could be used to treat wounds and vascular injuries (Steinbach & Husain, 2016).
Umbilical Cord Stem Cells in Regenerative Medicine
Umbilical cord stem cells (UCSCs) are a fantastic and versatile resource in regenerative medicine. One of the significant advantages of UCSCs is the ease with which they can be obtained. During childbirth, the umbilical cord is typically discarded despite containing a wealth of valuable stem cells that can be collected without harming the baby or the mother. This non-invasive and ethical procurement method distinguishes UCSCs from the more controversial stem cells. UCSCs are particularly rich in two types of stem cells: hematopoietic and mesenchymal stem cells (Shahrokhi et al., 2012). In addition to the ease of procuring UCSCs, they can be cryopreserved in stem cell banks. These banks can be operated by both the public and private sectors, each with a unique donation format. Public banks usually operate on a donation basis and conduct rigorous screening to ensure donated UCSCs are compatible with potential recipients. Private banks offer a more personalised approach, making cells available according to donor consent (Brown et al., 2019).

One of the notable aspects of UCSCs is their potential to address a wide range of medical conditions. UCSCs have received approval from the US Food and Drug Administration to treat various blood and immunological complications (Rosemann, 2014). UCSCs have shown promise in the treatment of various types of diabetes. For example, UCSCs can be applied in various regenerative therapies for conditions like systemic lupus erythematosus, which affects multiple organs and tissues (Cheng et al., 2020). The regenerative prospect of UCSCs extends to treating neurological diseases and injuries, such as Alzheimer's disease, Parkinson’s disease, amyotrophic lateral sclerosis, traumatic brain injuries, and stroke. Studies have shown that transplanted UCSCs can migrate to the central nervous system and peripheral organs (Ehrhart et al., 2016). They have also been explored for applications ranging from repairing tendons to regenerating cartilage and ligaments (Lv et al., 2021). UCSCs are even being investigated to treat hypertension, peritoneal fibrosis, and other inflammatory conditions (Fu et al., 2015). Moreover, ongoing research explores the use of UCSCs for addressing conditions like premature ovarian failure (POF), offering hope for non-invasive and effective treatments for women affected by POF (Shareghi-Oskoue et al., 2021). UCSCs are poised to revolutionise the field of regenerative medicine. They are an extraordinary resource of stem cells that are easy to collect, ethically sound and have been approved for treating various medical conditions.
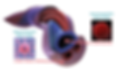
iPSCs in Regenerative Medicine
The emergence of induced pluripotent stem cells sent ripples of excitement throughout the scientific community. In 2006, a significant milestone was achieved by scientists Takahashi and Yamanaka when they successfully reprogrammed adult skin fibroblasts into cells that strikingly resembled embryonic stem cells. As previously explored in this 101 series, this remarkable feat was accomplished by introducing four specific genetic factors: Sox2, Oct3/4, Klf4, and c-Myc (Takahashi & Yamanaka, 2006). The continuous evolution of iPSC technology has significantly broadened its scope of applications. Today, researchers can generate iPSCs from a diverse range of adult cell types, either by passing through stages resembling embryonic stem cells or by direct reprogramming (Ballester et al., 2019). Recent breakthroughs have illuminated the vast potential of iPSCs in regenerative medicine. For instance, iPSCs can be harnessed to create kidney organoids that closely mimic the structure and function of real kidney tissue. This development holds immense promise for treating kidney injuries and diseases, potentially reshaping the landscape of kidney healthcare (Takasato et al., 2015).

When it comes to eye care, iPSCs have shown exceptional promise. They have demonstrated remarkable efficacy in addressing age-related macular degeneration, a leading cause of blindness. Through transplantation of iPSC-derived neuronal progenitor cells (NPCs), researchers have successfully halted the progression of ARMD in preclinical studies. These NPCs can potentially restore visual acuity, offering hope to those grappling with this condition (Tsai et al., 2015). Furthermore, iPSC technology holds significant potential for studying and treating neurodegenerative diseases. Scientists have managed to generate gamma-aminobutyric acid-secreting interneurons from iPSCs. When transplanted, these cells could be key in combating neurodegeneration and managing seizures (Colasante et al., 2015). Advancements also extend to creating miniature brain organoids from iPSCs, providing unique insights into autism spectrum disorder and schizophrenia (Mariani et al., 2015). iPSC-derived lung organoids have revolutionised the study of lung diseases, including tuberculosis and fibrosis, while also enabling the screening of potential drug treatments (Bosáková et al., 2022). iPSCs can potentially yield clinical-grade beta-cells, opening the door to more effective treatments for type 1 and type 2 diabetes (Zhu et al., 2016). One particularly intriguing possibility lies in the transplantation of iPSCs into closely related primate species to generate human organs. This innovative approach could provide a potential avenue for treating genetic diseases at the embryonic level (Mascetti & Pedersen, 2016).

Conclusions
The field of regenerative medicine is witnessing an impressive evolution, and at the heart of this transformation are different types of stem cells, each offering unique advantages and potential applications. Embryonic stem cells have long been at the forefront, holding immense regenerative promise with their pluripotency. Their versatility in differentiating into a wide array of cell types and tissues has positioned them as invaluable resources for addressing various medical conditions. However, ethical and sourcing concerns have tempered their progress. The ethical considerations surrounding ESCs have driven the exploration of UCSCs and iPSCs, broadening the field's horizons. Umbilical cord stem cells have appeared as a standout player in regenerative medicine. Especially rich in hematopoietic and mesenchymal stem cells, they have received approval for treating blood and immunological complications, diabetes, and other conditions. Induced pluripotent stem cells represent a groundbreaking era in stem cell research. Their creation, inspired by ESCs, has paved the way for reprogramming adult cells into a pluripotent state, offering regenerative hope across multiple disciplines. The prospect of generating human organs in closely related primates could reshape the treatment of genetic diseases. As demonstrated earlier in the series, other stem cell types exist, each with a distinctive potential for regenerative medicine. Thanks to diverse stem cell sources, regenerative medicine is on the cusp of remarkable breakthroughs. While challenges remain, including safety concerns in transplantation applications, the potential for addressing many medical conditions is a beacon of hope. As the scientific community continues to push boundaries, regenerative medicine promises to transform modern healthcare and offer new avenues for personalised treatments.
Bibliographical References
Acharya, M. M., Martirosian, V., Chmielewski, N. N., Hanna, N., Tran, K. K., Liao, A. C., Christie, L. A., Parihar, V. K., & Limoli, C. L. (2015). Stem cell transplantation reverses chemotherapy-induced cognitive dysfunction. Cancer Research, 75(4), 676–686. https://doi.org/10.1158/0008-5472.CAN-14-2237
Asch, D. A., & Volpp, K. G. (2017). Reimagining halfway technologies with behavioral science. Annals of Internal Medicine, 167(7), 505–506. https://doi.org/10.7326/M17-1449
Avior, Y., Levy, G., Zimerman, M., Kitsberg, D., Schwartz, R., Sadeh, R., Moussaieff, A., Cohen, M., Itskovitz-Eldor, J., & Nahmias, Y. (2015). Microbial-derived lithocholic acid and vitamin K2 drive the metabolic maturation of pluripotent stem cells-derived and fetal hepatocytes. Hepatology, 62(1), 265–278. https://doi.org/10.1002/hep.27803
Ballester, M., Bolonio, M., Santamaria, R., Castell, J. V., Ribes-Koninckx, C., & Bort, R. (2019). Direct conversion of human fibroblast to hepatocytes using a single inducible polycistronic vector. Stem Cell Research and Therapy, 10(1), 1–10. https://doi.org/10.1186/s13287-019-1416-5
Baraniak, P. R., & McDevitt, T. C. (2010). Stem cell paracrine actions and tissue regeneration. Regenerative Medicine, 5(1), 121–143. https://doi.org/10.2217/rme.09.74
Bosáková, V., De Zuani, M., Sládková, L., Garlíková, Z., Jose, S. S., Zelante, T., Hortová Kohoutková, M., & Frič, J. (2022). Lung organoids—the ultimate tool to dissect pulmonary diseases? Frontiers in Cell and Developmental Biology, 10. https://doi.org/10.3389/fcell.2022.899368
Bozek, D. N., Dunn, T. B., Kuhr, C. S., Marsh, C. L., Rogers, J., Rees, S. E., Basagoitia, L., Brunner, R. J., Roth, A. E., Ekwenna, O., Fumo, D. E., Krawiec, K. D., Kopke, J. E., Sindhwani, P., Ortiz, J., Tan, M., Paloyo, S. R., Punch, J. D., & Rees, M. A. (2018). Complete chain of the first global kidney exchange transplant and 3-yr follow-up. European Urology Focus, 4(2), 190–197. https://doi.org/10.1016/j.euf.2018.07.021
Brown, K. S., Rao, M. S., & Brown, H. L. (2019). The future state of newborn stem cell banking. Journal of Clinical Medicine, 8(1). https://doi.org/10.3390/jcm8010117
Bruin, J. E., Saber, N., Braun, N., Fox, J. K., Mojibian, M., Asadi, A., Drohan, C., O’Dwyer, S., Rosman-Balzer, D. S., Swiß, V. A., Rezania, A., & Kieffer, T. J. (2015). Treating diet-induced diabetes and obesity with human embryonic stem cell-derived pancreatic progenitor cells and antidiabetic drugs. Stem Cell Reports, 4(4), 605–620. https://doi.org/10.1016/j.stemcr.2015.02.011
Cheng, A., Kapacee, Z., Peng, J., Lu, S., Lucas, R. J., Hardingham, T. E., & Kimber, S. J. (2014). Cartilage repair using Human embryonic stem cell-derived chondroprogenitors. Stem Cells Translational Medicine, 3(11), 1287–1294. https://doi.org/10.5966/sctm.2014-0101
Cheng, T., Ding, S., Liu, S., Li, Y., & Sun, L. (2020). Human umbilical cord-derived mesenchymal stem cell therapy ameliorates lupus through increasing CD4+ T cell senescence via MiR-199a-5p/Sirt1/p53 axis. Theranostics, 11(2), 893–905. https://doi.org/10.7150/thno.48080
Colasante, G., Lignani, G., Rubio, A., Medrihan, L., Yekhlef, L., Sessa, A., Massimino, L., Giannelli, S. G., Sacchetti, S., Caiazzo, M., Leo, D., Alexopoulou, D., Dell’Anno, M. T., Ciabatti, E., Orlando, M., Studer, M., Dahl, A., Gainetdinov, R. R., Taverna, S., … Broccoli, V. (2015). Rapid conversion of fibroblasts into functional forebrain GABAergic interneurons by direct genetic reprogramming. Cell Stem Cell, 17(6), 719–734. https://doi.org/10.1016/j.stem.2015.09.002
Condic, M. L. (2014). Totipotency: What it is and what it is not. Stem Cells and Development, 23(8), 796–812. https://doi.org/10.1089/scd.2013.0364
Cronk, S. M., Kelly-Goss, M. R., Ray, H. C., Mendel, T. A., Hoehn, K. L., Bruce, A. C., Dey, B. K., Guendel, A. M., Tavakol, D. N., Herman, I. M., Peirce, S. M., & Yates, P. A. (2015). Adipose-derived stem cells from diabetic mice show impaired vascular stabilization in a murine model of diabetic retinopathy. Stem Cells Translational Medicine, 4(5), 459–467. https://doi.org/10.5966/sctm.2014-0108
D’Addio, F., Vasquez, A. V., Nasr, M. Ben, Franek, E., Zhu, D., Li, L., Ning, G., Snarski, E., & Fiorina, P. (2014). Autologous nonmyeloablative hematopoietic stem cell transplantation in new-onset type 1 diabetes: A multicenter analysis. Diabetes, 63(9), 3041–3046. https://doi.org/10.2337/db14-0295
De Miguel, M. P., Prieto, I., Moratilla, A., Arias, J., & Aller, M. A. (2019). Mesenchymal stem cells for liver regeneration in liver failure: From experimental models to clinical trials. Stem Cells International, 2019. https://doi.org/10.1155/2019/3945672
Edgar, L., Pu, T., Porter, B., Aziz, J. M., La Pointe, C., Asthana, A., & Orlando, G. (2020). Regenerative medicine, organ bioengineering and transplantation. British Journal of Surgery, 107(7), 793–800. https://doi.org/10.1002/bjs.11686
Ehrhart, J., Darlington, D., Kuzmin-Nichols, N., Sanberg, C. D., Sawmiller, D. R., Sanberg, P. R., & Tan, J. (2016). Biodistribution of infused human umbilical cord blood cells in Alzheimer’s disease-like murine model. Cell Transplantation, 25(1), 195–199. https://doi.org/10.3727/096368915X689604
Fernandes, S., Chong, J. J. H., Paige, S. L., Iwata, M., Torok-Storb, B., Keller, G., Reinecke, H., & Murry, C. E. (2015). Comparison of human embryonic stem cell-derived cardiomyocytes, cardiovascular progenitors, and bone marrow mononuclear cells for cardiac repair. Stem Cell Reports, 5(5), 753–762. https://doi.org/10.1016/j.stemcr.2015.09.011
Fu, L., Liu, Y., Zhang, D., Xie, J., Guan, H., & Shang, T. (2015). Beneficial effect of human umbilical cord‑derived mesenchymal stem cells on an endotoxin‑induced rat model of preeclampsia. Experimental and Therapeutic Medicine, 10(5), 1851–1856. https://doi.org/10.3892/etm.2015.2742
Fuoco, C., Rizzi, R., Biondo, A., Longa, E., Mascaro, A., Shapira‐Schweitzer, K., Kossovar, O., Benedetti, S., Salvatori, M. L., Santoleri, S., Testa, S., Bernardini, S., Bottinelli, R., Bearzi, C., Cannata, S. M., Seliktar, D., Cossu, G., & Gargioli, C. (2015). In vivo generation of a mature and functional artificial skeletal muscle. EMBO Molecular Medicine, 7(4), 411–422. https://doi.org/10.15252/emmm.201404062
Garzón, I., Pérez-Köhler, B., Garrido-Gómez, J., Carriel, V., Nieto-Aguilar, R., Martín-Piedra, M. A., García-Honduvilla, N., Buján, J., Campos, A., & Alaminos, M. (2012). Evaluation of the cell viability of human Wharton’s Jelly stem cells for use in cell therapy. Tissue Engineering - Part C: Methods, 18(6), 408–419. https://doi.org/10.1089/ten.tec.2011.0508
Greggio, C., De Franceschi, F., Figueiredo-Larsen, M., Gobaa, S., Ranga, A., Semb, H., Lutolf, M., & Grapin-Botton, A. (2013). Artificial three-dimensional niches deconstruct pancreas development in vitro. Development (Cambridge), 140(21), 4452–4462. https://doi.org/10.1242/dev.096628
Gubareva, E. A., Sjöqvist, S., Gilevich, I. V., Sotnichenko, A. S., Kuevda, E. V., Lim, M. L., Feliu, N., Lemon, G., Danilenko, K. A., Nakokhov, R. Z., Gumenyuk, I. S., Grigoriev, T. E., Krasheninnikov, S. V., Pokhotko, A. G., Basov, A. A., Dzhimak, S. S., Gustafsson, Y., Bautista, G., Beltrán Rodríguez, A., … Macchiarini, P. (2016). Orthotopic transplantation of a tissue engineered diaphragm in rats. Biomaterials, 77, 320–335. https://doi.org/10.1016/j.biomaterials.2015.11.020
Hargraves, I. G., Behfar, A., Foxen, J. L., Montori, V. M., & Terzic, A. (2018). Towards regeneration: The evolution of medicine from fighting to building. BMJ (Online), 361. https://doi.org/10.1136/bmj.k1586
Hofmann, M., Wollert, K. C., Meyer, G. P., Menke, A., Arseniev, L., Hertenstein, B., Ganser, A., Knapp, W. H., & Drexler, H. (2005). Monitoring of bone marrow cell homing into the infarcted human myocardium. Circulation, 111(17), 2198–2202. https://doi.org/10.1161/01.CIR.0000163546.27639.AA
Hogan, M. S., Parfitt, D. E., Zepeda-Mendoza, C. J., Shen, M. M., & Spector, D. L. (2015). Transient pairing of homologous Oct4 alleles accompanies the onset of embryonic stem cell differentiation. Cell Stem Cell, 16(3), 275–288. https://doi.org/10.1016/j.stem.2015.02.001
Ksander, B. R., Kolovou, P. E., Wilson, B. J., Saab, K. R., Guo, Q., Ma, J., McGuire, S. P., Gregory, M. S., Vincent, W. J. B., Perez, V. L., Cruz-Guilloty, F., Kao, W. W. Y., Call, M. K., Tucker, B. A., Zhan, Q., Murphy, G. F., Lathrop, K. L., Alt, C., Mortensen, L. J., … Frank, N. Y. (2014). ABCB5 is a limbal stem cell gene required for corneal development and repair. Nature, 511(7509), 353–357. https://doi.org/10.1038/nature13426
Kucia, M., Shin, D. M., Liu, R., Ratajczak, J., Bryndza, E., Masternak, M. M., Bartke, A., & Ratajczak, M. Z. (2011). Reduced number of VSELs in the bone marrow of growth hormone transgenic mice indicates that chronically elevated Igf1 level accelerates age-dependent exhaustion of pluripotent stem cell pool: A novel view on aging. Leukemia, 25(8), 1370–1374. https://doi.org/10.1038/leu.2011.98
Lv, X., Wang, L., Zou, X., & Huang, S. (2021). Umbilical cord mesenchymal stem cell therapy for regenerative treatment of rheumatoid arthritis: Opportunities and challenges. Drug Design, Development and Therapy, 15, 3927–3936. https://doi.org/10.2147/DDDT.S323107
Mahla, R. S. (2016). Stem cells applications in regenerative medicine and disease therapeutics. International Journal of Cell Biology, 2016. https://doi.org/10.1155/2016/6940283
Mariani, J., Coppola, G., Zhang, P., Abyzov, A., Provini, L., Tomasini, L., Amenduni, M., Szekely, A., Palejev, D., Wilson, M., Gerstein, M., Grigorenko, E. L., Chawarska, K., Pelphrey, K. A., Howe, J. R., & Vaccarino, F. M. (2015). FOXG1-dependent dysregulation of GABA/glutamate neuron differentiation in autism spectrum disorders. Cell, 162(2), 375–390. https://doi.org/10.1016/j.cell.2015.06.034
Mascetti, V. L., & Pedersen, R. A. (2016). Human-mouse chimerism validates human stem cell pluripotency. Cell Stem Cell, 18(1), 67–72. https://doi.org/10.1016/j.stem.2015.11.017
Mason, C., & Dunnill, P. (2008). A brief definition of regenerative medicine. Regenerative Medicine, 3(1), 1–5. https://doi.org/10.2217/17460751.3.1.1
Massie, A. B., Kuricka, L. M., & Segev, D. L. (2014). Big data in organ transplantation: Registries and administrative claims. American Journal of Transplantation, 14(8), 1723–1730. https://doi.org/10.1111/ajt.12777
Mizutari, K., Fujioka, M., Hosoya, M., Bramhall, N., Okano, H. J., Okano, H., & Edge, A. S. B. (2013). Notch inhibition induces cochlear hair cell regeneration and recovery of hearing after acoustic trauma. Neuron, 77(1), 58–69. https://doi.org/10.1016/j.neuron.2012.10.032
Nathamgari, S. S. P., Dong, B., Zhou, F., Kang, W., Giraldo-Vela, J. P., McGuire, T., McNaughton, R. L., Sun, C., Kessler, J. A., & Espinosa, H. D. (2015). Isolating single cells in a neurosphere assay using inertial microfluidics. Lab on a Chip, 15(24), 4591–4597. https://doi.org/10.1039/c5lc00805k
Orlando, G., Baptista, P., Birchall, M., De Coppi, P., Farney, A., Guimaraes-Souza, N. K., Opara, E., Rogers, J., Seliktar, D., Shapira-Schweitzer, K., Stratta, R. J., Atala, A., Wood, K. J., & Soker, S. (2011). Regenerative medicine as applied to solid organ transplantation: Current status and future challenges. Transplant International, 24(3), 223–232. https://doi.org/10.1111/j.1432-2277.2010.01182.x
Orlando, G., Wood, K. J., Soker, S., & Stratta, R. J. (2011). How regenerative medicine may contribute to the achievement of an immunosuppression-free state. Transplantation, 92(8). https://doi.org/10.1097/TP.0b013e31822f59d8
Petersdorf, E. W., Malkki, M., Gooley, T. A., Martin, P. J., & Guo, Z. (2007). MHC haplotype matching for unrelated hematopoietic cell transplantation. PLoS Medicine, 4(1), 0059–0068. https://doi.org/10.1371/journal.pmed.0040008
Potdar, P. D. (2015). Human dental pulp stem cells: Applications in future regenerative medicine. World Journal of Stem Cells, 7(5), 839. https://doi.org/10.4252/wjsc.v7.i5.839
Ratajczak, M. Z., Bujko, K., & Wojakowski, W. (2016a). Stem cells and clinical practice: New advances and challenges at the time of emerging problems with induced pluripotent stem cell therapies. Polskie Archiwum Medycyny Wewnetrznej, 126(11), 879–890. https://doi.org/10.20452/pamw.3644
Ratajczak, M. Z., Bujko, K., & Wojakowski, W. (2016b). Stem cells and clinical practice: New advances and challenges at the time of emerging problems with induced pluripotent stem cell therapies. Polskie Archiwum Medycyny Wewnetrznej, 126(11), 879–890. https://doi.org/10.20452/pamw.3644
Rosemann, A. (2014). Why regenerative stem cell medicine progresses slower than expected. Journal of Cellular Biochemistry 115(12), 2073–2076). https://doi.org/10.1002/jcb.24894
Shahrokhi, S., Menaa, F., Alimoghaddam, K., McGuckin, C., & Ebtekar, M. (2012). Insights and hopes in umbilical cord blood stem cell transplantations. Journal of Biomedicine and Biotechnology 2012. https://doi.org/10.1155/2012/572821
Shareghi-Oskoue, O., Aghebati-Maleki, L., & Yousefi, M. (2021). Transplantation of human umbilical cord mesenchymal stem cells to treat premature ovarian failure. Stem Cell Research and Therapy 12(1), 454). https://doi.org/10.1186/s13287-021-02529-w
Shroff, G., & Gupta, R. (2015). Human embryonic stem cells in the treatment of patients with spinal cord injury. Annals of Neurosciences, 22(4), 208–216. https://doi.org/10.5214/ans.0972.7531.220404
Simon, L., Ekman, G. C., Kostereva, N., Zhang, Z., Hess, R. A., Hofmann, M. C., & Cooke, P. S. (2009). Direct transdifferentiation of stem/progenitor spermatogonia into reproductive and nonreproductive tissues of all germ layers. Stem Cells, 27(7), 1666–1675. https://doi.org/10.1002/stem.93
Steinbach, S. K., & Husain, M. (2016). Vascular smooth muscle cell differentiation from human stem/progenitor cells. Methods 101, 85–92. Methods. https://doi.org/10.1016/j.ymeth.2015.12.004
Suman, S., Domingues, A., Ratajczak, J., Ratajczak, M.Z. (2019). Potential Clinical Applications of Stem Cells in Regenerative Medicine. In: Ratajczak, M. (Ed) Stem Cells. Advances in Experimental Medicine and Biology, 1201. Springer, Cham. https://doi.org/10.1007/978-3-030-31206-0_1
Takahashi, K., & Yamanaka, S. (2006). Induction of pluripotent stem cells from mouse embryonic and adult fibroblast cultures by defined factors. Cell, 126(4), 663–676. https://doi.org/10.1016/j.cell.2006.07.024
Takasato, M., Er, P. X., Chiu, H. S., Maier, B., Baillie, G. J., Ferguson, C., Parton, R. G., Wolvetang, E. J., Roost, M. S., De Sousa Lopes, S. M. C., & Little, M. H. (2015). Kidney organoids from human iPS cells contain multiple lineages and model human nephrogenesis. Nature, 526(7574), 564–568. https://doi.org/10.1038/nature15695
Terzic, A., & Waldman, S. (2011). Chronic diseases: The emerging pandemic. Clinical and Translational Science 4(3), 225–226. https://doi.org/10.1111/j.1752-8062.2011.00295.x
Thompson, P. A., Perera, T., Marin, D., Oran, B., Popat, U., Qazilbash, M., Shah, N., Parmar, S., Rezvani, K., Olson, A., Kebriaei, P., Anderlini, P., Rondon, G., Alousi, A., Ciurea, S., Champlin, R. E., Bajel, A., Szer, J., Shpall, E. J., … Hosing, C. M. (2016). Double umbilical cord blood transplant is effective therapy for relapsed or refractory Hodgkin lymphoma. Leukemia and Lymphoma, 57(7), 1607–1615. https://doi.org/10.3109/10428194.2015.1105370
Thomson, J. A. (1998). Embryonic stem cell lines derived from human blastocysts. Science, 282(5391), 1145–1147. https://doi.org/10.1126/science.282.5391.1145
Tsai, Y., Lu, B., Bakondi, B., Girman, S., Sahabian, A., Sareen, D., Svendsen, C. N., & Wang, S. (2015). Human iPSC-derived neural progenitors preserve vision in an AMD-like model. Stem Cells, 33(8), 2537–2549. https://doi.org/10.1002/stem.2032
Vedantham, V. (2015). New approaches to biological pacemakers: Links to sinoatrial node development. Trends in Molecular Medicine 21(12), 49–761. https://doi.org/10.1016/j.molmed.2015.10.002
Vincenti, F., Rostaing, L., Grinyo, J., Rice, K., Steinberg, S., Gaite, L., Moal, M.-C., Mondragon-Ramirez, G. A., Kothari, J., Polinsky, M. S., Meier-Kriesche, H.-U., Munier, S., & Larsen, C. P. (2016). Belatacept and long-term outcomes in kidney transplantation. New England Journal of Medicine, 374(4), 333–343. https://doi.org/10.1056/nejmoa1506027
Visvader, J. E., & Clevers, H. (2016). Tissue-specific designs of stem cell hierarchies. Nature Cell Biology 18(4), 349–355. https://doi.org/10.1038/ncb3332
Wells, J. M., & Watt, F. M. (2018). Diverse mechanisms for endogenous regeneration and repair in mammalian organs. Nature 557(7705), 322–328. https://doi.org/10.1038/s41586-018-0073-7
Yamada, S., Behfar, A., & Terzic, A. (2021a). Regenerative medicine clinical readiness. Regenerative Medicine, 16(3), 309–322. https://doi.org/10.2217/rme-2020-0178
Yamada, S., Behfar, A., & Terzic, A. (2021b). Regenerative medicine clinical readiness. Regenerative Medicine 16(3), 309–322. https://doi.org/10.2217/rme-2020-0178
Ye, L., Robertson, M. A., Mastracci, T. L., & Anderson, R. M. (2016). An insulin signaling feedback loop regulates pancreas progenitor cell differentiation during islet development and regeneration. Developmental Biology 409(2), 354–369. https://doi.org/10.1016/j.ydbio.2015.12.003
Zakrzewski, W., Dobrzyński, M., Szymonowicz, M., & Rybak, Z. (2019). Fuel cells: Past, present and future. Stem Cell Research and Therapy, 128(5), 329–332. https://doi.org/10.1541/ieejfms.128.329
Zhou, S., Flamier, A., Abdouh, M., Tétreault, N., Barabino, A., Wadhwa, S., & Bernier, G. (2015). Differentiation of human embryonic stem cells into cone photoreceptors through simultaneous inhibition of BMP, TGFβ and Wnt signaling. Development (Cambridge), 142(19), 3294–3306. https://doi.org/10.1242/dev.125385
Zhu, S., Russ, H. A., Wang, X., Zhang, M., Ma, T., Xu, T., Tang, S., Hebrok, M., & Ding, S. (2016). Human pancreatic beta-like cells converted from fibroblasts. Nature Communications, 7(1), 1–13. https://doi.org/10.1038/ncomms10080
Visual Sources
Cover Image: [An image alluding to a futuristic lab]. (n.d.). [Illustration]. SoCal Regenerative Medical Clinics. https://www.socalregenclinic.com/is-regenerative-medicine-the-future-of-medicine/
Figure 1: Regenerative medicine perspective. Yamada, S., Behfar, A., & Terzic, A. (2021b). [Illustration]. Regenerative medicine clinical readiness. In Regenerative Medicine (Vol. 16, Issue 3, pp. 309–322). Future Science Group. https://doi.org/10.2217/rme-2020-0178
Figure 2: Regenerative medicine aspiration. Yamada, S., Behfar, A., & Terzic, A. (2021b). [Illustration]. Regenerative medicine clinical readiness. In Regenerative Medicine (Vol. 16, Issue 3, pp. 309–322). Future Science Group. https://doi.org/10.2217/rme-2020-0178
Figure 3: Overall goals of regenerative medicine. Ratajczak, M. (2019). [Illustration]. Stem cells : Therapeutic applications. https://doi.org/10.1007/978-3-030-31206-0
Figure 4: Stem cells overview. (n.d.). [Illustration]. Novus Biologicals. https://www.novusbio.com/research-areas/stem-cells
Figure 5: The tree of stem cell differentiation. (n.d.). [Illustration]. Arigo Biolaboratories. https://www.arigobio.com/news/stem-cell-and-the-regenerative-medicine-ready-for-the-patients
Figure 6: Phases in the history of organ transplantation. Edgar, L., Pu, T., Porter, B., Aziz, J. M., La Pointe, C., Asthana, A., & Orlando, G. (2020). [Illustration]. Regenerative medicine, organ bioengineering and transplantation. In British Journal of Surgery (Vol. 107, Issue 7, pp. 793–800). Br J Surg. https://doi.org/10.1002/bjs.11686
Figure 7: Promises of stem cells in regenerative medicine. Mahla, R. S. (2016). [Illustration]. Stem cells applications in regenerative medicine and disease therapeutics. In International Journal of Cell Biology (Vol. 2016). https://doi.org/10.1155/2016/6940283
Figure 8: Mesenchymal stem cells and their potential. de Fazio, S., Lucattelli, E. (2022). [Illustration]. Regenerative surgery: Definitions and background. In: Kalaaji, A. (eds) Plastic and aesthetic regenerative surgery and fat grafting. Springer, Cham. https://doi.org/10.1007/978-3-030-77455-4_3
Figure 9: ESCs in regenerative medicine. Mahla, R. S. (2016). [Illustration]. Stem cells applications in regenerative medicine and disease therapeutics. In International Journal of Cell Biology (Vol. 2016). https://doi.org/10.1155/2016/6940283
Figure 10: Global human embryonic stem cell market. (n.d.). EMR. https://www.expertmarketresearch.com/reports/human-embryonic-stem-cell-market
Figure 11: TSPSCs in regenerative medicine. Mahla, R. S. (2016). [Illustration]. Stem cells applications in regenerative medicine and disease therapeutics. In International Journal of Cell Biology (Vol. 2016). https://doi.org/10.1155/2016/6940283
Figure 12: Modulating the stem cell niche is crucial for regeneration. Lane, S., Williams, D. & Watt, F. Modulating the stem cell niche for tissue regeneration. Nat Biotechnol32, 795–803 (2014). [Illustration]. https://doi.org/10.1038/nbt.2978
Figure 13: UCSCs in regenerative medicine. Mahla, R. S. (2016). [Illustration]. Stem cells applications in regenerative medicine and disease therapeutics. In International Journal of Cell Biology (Vol. 2016). https://doi.org/10.1155/2016/6940283
Figure 14: The umbilical cord. (n.d.). [Infographic]. Health Biotec. https://www.health-biotech.fr/en/technology/umbilical-cord-stem-cells/
Figure 15: iPSCs in regenerative medicine. Mahla, R. S. (2016). [Illustration]. Stem cells applications in regenerative medicine and disease therapeutics. In International Journal of Cell Biology (Vol. 2016). https://doi.org/10.1155/2016/6940283
Figure 16: iPSCs - overview. (n.d.). [Infographic]. Bayer. https://www.bayer.com/en/pharma/stem-cells-new-age-medical-research