Stem Cell Biotechnology 101: Sources and Isolation Methods
Foreword
The field of stem cells is brimming with promise and intrigue. As the understanding of these remarkable foundational components of life continues to evolve, so does the capacity to harness their extraordinary potential. Stem cells have enchanted the minds of scientists, medical professionals, and curious individuals alike, presenting unparalleled opportunities to revolutionise healthcare and regenerative medicine. This series of articles aims to unravel the mysteries of stem cell differentiation, explore the mechanisms governing their self-renewal, and examine the factors that shape their destiny. Within these articles, readers may discover the immense potential of induced pluripotent stem cells in reshaping personalised medicine while uncovering their awe-inspiring regenerative capabilities. Moreover, this series sheds light on the critical challenges and limitations inherently intertwined with this field, from ensuring the safety and efficacy of stem cell therapies through navigating the complex terrain of regulatory frameworks and public perception to debating ethical uncertainties.
This 101 series is divided into eight articles, including:
Stem Cell Biotechnology 101: Exploring the Potential of Stem Cells
Stem Cell Biotechnology 101: Sources and Isolation Methods
Stem Cell Biotechnology 101: Differentiation and Lineage Commitment
Stem Cell Biotechnology 101: Self-Renewal and Maintenance
Stem Cell Biotechnology 101: Induced Pluripotent Stem Cells (iPSCs) and Genetic Engineering
Stem Cell Biotechnology 101: Stem Cells in Regenerative Medicine
Stem Cell Biotechnology 101: Stem Cells in Disease Modelling and Cancer Research
Stem Cell Biotechnology 101: Ethical Considerations and Future Perspectives
Stem Cell Biotechnology 101: Sources and Isolation Methods
Introduction
As explained in the previous article, the significance of stem cells in medicine is immense. Their regenerative capabilities, self-renewal and multi-lineage differentiation hold promise for treating conditions thought of as incurable. Stem cells are the fundamental building blocks responsible for organ development and tissue regeneration. They can be obtained from different sources, such as embryonic tissue, foetal tissues, adult tissues, genetically reprogrammed somatic cells and induced pluripotent stem cells. The isolation techniques employed to obtain these diverse stem cell populations each come with their advantages and limitations. This article will delve into these isolation methods, explore the various sources of stem cells, and provide additional insights into the different types of stem cells available for research and clinical applications.
Insight Into Stem Cell Types and Their Harvest
To provide a comprehensive understanding for readers who may be encountering this topic for the first time, the five categories of stem cells are as listed: embryonic stem cells (ESCs), very small embryonic-like stem cells (VSELs), induced pluripotent stem cells (iPSCs), nuclear transfer stem cells (NTSCs), and adult stem cells (ASCs). (G. Liu et al., 2020).
Embryonic Stem Cells and Nuclear Transfer Stem Cells

Embryonic stem cells (ESCs) are derived from early-stage blastocysts, typically around 4 to 5 days post-fertilisation (G. Liu et al., 2020). Isolating these cells and creating immortalised embryonic stem cell lines under suitable culture conditions can be challenging. Obtaining ESCs involves the destruction of the source blastocyst; in other words, they can be acquired from cryopreserved unused embryos in IVF clinics, which has raised ethical concerns (Landry & Zucker, 2004). ESCs can also be harvested from later-stage tissues, typically from embryos at a gestational age of 3 months or less. Additionally, the unique combination of histocompatibility genes inherited from the sperm and egg in these cells poses a challenge for transplantation, as histoincompatible recipients may reject them. Finding a suitable donor match becomes crucial in such cases (English & Wood, 2011). ESCs were among the first stem cells to be extensively studied and applied in research settings. They have played a crucial role in advancing the understanding of stem cell biology and its potential applications. Currently, ESCs are still commonly used in clinical trials, demonstrating their ongoing significance in various areas of medical research, even though controlling ESCs is difficult, as they have the potential to form teratomas, and the process of deriving fully functional differentiated somatic cells from them still presents a notable obstacle (Storchová, 2016).
An alternative approach involves the generation of immortalised ESC lines through a process known as therapeutic cloning. Initially, this strategy entails the transfer of a nucleus obtained from a patient-derived somatic cell into an enucleated oocyte procured from a female donor. The patient's nucleus, devoid of its differentiated state, undergoes dedifferentiation within the oocyte's cytoplasm, facilitated by various enzymes, proteins, mRNAs, and miRNAs (Qin et al., 2015). This dedifferentiated state resembles the nucleus found in ESCs. The resulting artificially created stem cells, referred to as clonotes, have the potential to develop into blastocysts from which ESC lines can be derived. Importantly, since the patient's nucleus contains all the histocompatibility genes, it becomes possible to generate ‘customised’ ESCs that may not trigger an immune response from the patient. However, ethical concerns and the risk of karyotypic abnormalities persist, and they parallel the challenges encountered with ESCs derived from naturally fertilised blastocysts. Due to moral uneasiness and technical obstacles, further advancements in this potential source of pluripotent stem cells have been, for now, discontinued (Harris, 1997). It is crucial to note that the implantation of embryos created through nuclear transfer into the uterus can also lead to reproductive cloning, as exemplified by the successful cloning of Dolly the sheep (Wilmut et al., 1997).

Both ESC and NTSC have similar properties in that they can differentiate into different types of cells and potentially be used in medical research. However, their origins and methods of creation are different. It is crucial to distinguish between ESC and NTSC because the ethical considerations and controversies surrounding their use are distinct. ESC research involves embryos, which raises ethical questions for some. On the other hand, SCNT involves creating embryos through a specific laboratory technique (Gouveia et al., 2020).
Chinese scientists achieved the cloning of macaque monkeys using SCNT, opening up new possibilities for disease research and biomedical studies. Cloned primates offer genetically uniform animal models that can help investigate disease mechanisms and drug targets and even be used for genetic engineering experiments. The demand for cloned monkeys in pharmaceutical drug testing has led to establishing international primate research centres, demonstrating the potential and significance of SCNT in both basic research and clinical applications. Unlike other stem cell approaches, SCNT has the unique ability to generate an entire living body, making it advantageous for studying biophysiological functions (Z. Liu et al., 2018).

Although therapeutic cloning has been demonstrated in humans, the prospect of reproductive cloning has raised huge ethical dilemmas and public noise, not to mention the considerable risks involved (M. Ratajczak, 2019).
Very Small Embryonic-Like Stem Cells
Very Small Embryonic-Like Stem Cells (VSELs) were first identified in 2006 by Ratajczak et al. (M. Z. Ratajczak et al., 2006), and several independent laboratories have confirmed their existence (Bhartiya et al., 2016; Suszynska et al., 2014). Still, some groups have questioned their existence (Abbott, 2013; Danova-Alt et al., 2012). VSELs are small stem cells found in adult tissues that exhibit pluripotency markers and possess a primitive morphology and gene expression profile. Morphologically, VSELs resemble the cells found in the inner cell mass of the blastocyst and are approximately 3 to 5 μm in mice and 5 to 7 μm in humans (for reference, they are slightly smaller than red blood cells). They express markers associated with both ESCs and migrating primordial germ cells. The developmental origin of VSELs is believed to be associated with germ-line deposits during embryogenesis (G. Liu et al., 2020).
Recent studies have provided intriguing insights into the potential of ovary-isolated VSELs. These cells have demonstrated the ability to differentiate into oocyte-like cells upon exposure to sperm cells, initiating the fertilisation process. Notably, the quantity of VSELs has been found to correlate with longevity in specific long-living murine strains (Shin et al., 2010). Experimental approaches such as caloric restriction, regular exercise, and administration of DNA modifiers like nicotinamide or valproic acid have shown promise in increasing the number of VSELs in animal models. Conversely, increased insulin/insulin-like growth factor signalling has been associated with premature ageing and depletion of VSELs from tissues. Various studies have highlighted the contribution of injected purified VSELs to haematopoiesis, osteogenesis, and angiogenesis, as well as tissues like the myocardium, liver, and pulmonary alveolar epithelium in relevant in vivo models. Chimerism observed in several organs indicates the potential of VSELs to differentiate across germ layers (Mariusz Z. Ratajczak et al., 2008).

The discovery of practical ways to grow VSELs outside the body using substances like nicotinamide, valproic acid or UM177 is a significant advancement in studying and using these cells. These methods do not involve introducing DNA or RNA or using special supporting cells. By using nicotinamide or valproic acid to block a protein called sirtuin, the growth of VSELs can be promoted in the laboratory while keeping their unique abilities and preventing the formation of teratomas. This exciting progress has encouraged researchers to further investigate the potential of these cells isolated from adult tissues, especially with the improved techniques for growing them outside the body (M. Ratajczak, 2019).
Typically, the course of action for obtaining VSELs begins with tissue dissociation, starting with collecting adult tissue such as bone marrow or peripheral blood. The tissue is broken down into individual cells through enzymatic digestion or mechanical disruption. Enzymatic digestion involves treating the tissue with enzymes like collagenase or trypsin to break down the extracellular matrix and release the cells (Alstrup et al., 2019). Mechanical methods, such as grinding or homogenisation, physically disrupt the tissue to release the cells (Hosseini et al., 2020). After tissue dissociation, the resulting mixture contains various cell types, including VSELs. The cell suspension is filtered or centrifuged to remove larger debris to obtain a more homogeneous cell population (Qin et al., 2015).
The next step involves isolating the VSELs from the cell suspension using specific cell sorting techniques like fluorescence-activated cell sorting (FACS) or magnetic-activated cell sorting (MACS) (Bhartiya et al., 2019; Danova-Alt et al., 2012; Guenther et al., 2022). FACS enables high-speed and high-resolution sorting based on specific surface markers, isolating rare cell populations like VSELs. However, FACS requires specialised equipment and may introduce potential alterations in cellular behaviour. On the other hand, MACS provides a more straightforward and less expensive alternative by using magnetic beads coated with antibodies to isolate VSELs via a magnetic field. MACS may have lower resolution and purity compared to FACS but can be performed using standard laboratory equipment (Pan & Wan, 2020). Marker-based enrichment methods, utilising antibodies or probes specific to VSEL markers, can also enhance the VSEL population's purity. However, these methods usually require additional steps to obtain highly enriched VSEL samples. Once the VSELs have been sorted or selected, additional purification steps may be performed to increase the purity of the cell population. This step can involve repeating the sorting or selection process or using additional markers specific to VSELs to enrich the cell population further. These purification stages help obtain a more homogeneous population of VSELs for downstream applications (Mariusz Z. Ratajczak et al., 2008).
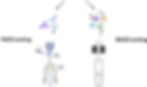
Induced Pluripotent Stem Cells
Induced pluripotent stem cells (iPSCs) have remained an incredibly topical issue since Yamanaka and colleagues' revolutionary report from 2006 (Takahashi & Yamanaka, 2006). Afterwards, the field of reprogramming technologies witnessed remarkable advancements. These innovations have opened up new possibilities, particularly in direct reprogramming methods both in vitro and in vivo, which enable the production of specific tissue lineages by manipulating lineage-restricted transcription factors, RNA signal modifications, and using small molecules or chemicals. This direct approach bypasses the intermediate step of iPSC generation. Consequently, it produces more refined cell types, such as induced neural progenitor cells, which exhibit a closer resemblance to the desired target cell lineage, such as neural cells and subsequent motor neurons (G. Liu et al., 2020).
The development of reprogrammed stem cells involves employing manual laboratory methods to reprogram the genetic signals of primary cells without employing the somatic cell nuclear transfer technique used in creating iPSCs. This alternative strategy is driven by the need to address ethical concerns associated with obtaining human ESCs by using human oocytes to create embryos. By isolating cells from normal adult tissues, such as skin fibroblasts, they offer a potential solution to overcome these ethical and immunogenic challenges associated with ESCs. Prominently, iPSCs derived from adult somatic tissues, referred to as human-induced pluripotent stem cells (hiPSCs), hold great promise in regenerative medicine. The advantage of hiPSCs lies in their abundant sources, including blood, skin, and urine, which can be readily accessed. Additionally, hiPSCs can be harvested from individual patients, allowing for autologous transplantation and reducing the risk of immune rejection, making them a valuable asset in personalised medicine (Ofir, 2021; Park et al., 2015).

Once the reprogramming factors are introduced, the cells undergo culture in specific conditions that facilitate their transition to a pluripotent state. This process entails activating genes associated with pluripotency while repressing genes specific to somatic cells. Over several weeks, the cells gradually acquire the characteristics of induced pluripotent stem cells (G. Liu et al., 2020). Following successful reprogramming, the subsequent step involves isolating and selecting iPSC colonies, typically accomplished through visual examination, where colonies display the distinctive morphology of iPSCs. These are compact and domed structures with well-defined edges. The selected colonies are then transferred to new culture plates or dishes to facilitate their further expansion (Bacakova et al., 2018).
Additional techniques can be employed to ensure the purity and quality of the iPSC population. Similarly to VSELs, these techniques include FACS and MACS. The choice of isolation technique is influenced by factors such as the availability of equipment and antibodies and the specific requirements of the experiment or application —as aforementioned, both FACS and MACS present advantages and limitations (Pan & Wan, 2020).

The versatility of hiPSCs is exemplified by their capacity to differentiate into a broad spectrum of tissues and cell lineages. Almost any mature cell type in the human body, ranging from umbilical cord blood cells, bone marrow cells, and peripheral blood cells to fibroblasts, keratinocytes, and even cells in the urine, can be reprogrammed into hiPSCs and subsequently differentiated into tissue-specific cells of desired lineages (Lukovic et al., 2017; M. Ratajczak, 2019).
Among the various sources, the isolation of hiPSCs from urine samples has emerged as a particularly attractive and practical approach. A groundbreaking protocol for obtaining hiPSCs from urine-derived renal tubular cells was introduced by Zhou et al. in 2011, followed by a detailed method for obtaining exfoliated renal epithelial cells published by the same group the following year. The simplicity, cost-effectiveness, and universality of this method have been demonstrated, as it only requires a small sample of urine (30 mL) and involves a relatively short period of cell culturing and reprogramming. The resulting urine-derived iPSCs have exhibited a normal karyotype and demonstrated the potential to differentiate into all three germ layers, as confirmed by teratoma assays (Zhou et al., 2012). Moreover, urine-derived cells have shown promise in various systems, including the cardiovascular system, where functional cardiomyocytes were generated following the differentiation of reprogrammed iPSCs using lentiviral-vector gene transduction (Sun et al., 2018).
Despite their immense potential, iPSCs have posed certain limitations. The presence of the Yamanaka factors, particularly c-Myc and Klf-4, which are potent oncogenes, has raised concerns regarding the risk of teratoma and cancer formation. Furthermore, integrating these genes into manipulated cells' chromosomes can trigger oncogenes' activation or inactivate tumour suppressor genes through insertional mutagenesis (Feng et al., 2009).

Although advancements in reprogramming and differentiation techniques hold great promise, it is crucial to carefully evaluate the behaviour of reprogrammed cells in culture and their performance in clinical studies. Genomic instability and variability inherent in iPSCs must be considered when interpreting data obtained from these cells. However, ongoing efforts to enhance the clinical safety of iPSCs, such as the use of self-destruction genes to eliminate undifferentiated iPSCs, the selection of mutation-free cell sources, and the utilisation of non-integrating vectors for reprogramming, provide avenues for mitigating potential risks associated with iPSC therapies (M. Ratajczak, 2019).
Considering the weight and relevance of iPSCs, they will be discussed in more depth in a separate article.
Adult Stem Cells
When adult stem cells (ASCs) were first discovered, they sparked excitement about their potential medical use (S. Liu et al., 2016). However, while hematopoietic stem cell transplants have shown promising results, stem cell therapies for other conditions have been disappointing in clinical outcomes. They can be obtained from different sources, including bone marrow, umbilical cord blood, mobilised peripheral blood, skin epithelium, myocardium, adipose tissues, and skeletal muscle biopsies. These stem cells in adult organs rely on signals in their microenvironment for their survival, quiescence, and activation. They can recognise damaged areas and regenerate missing cells. Tissue-resident ASCs are accessible sources for cell therapy as they can self-renew and differentiate into different cell types without immune rejection. Exogenous small molecules can also stimulate the growth and differentiation of existing cells in the body (Kuci et al., 2009).
Mesenchymal stem cells are the most widely used ASCs, obtained from various sources such as bone marrow, umbilical cord blood, adipose tissue, placenta, amniotic fluid, and menstrual blood. Each source has its concerns and standardised procedures. Stem cells from bone marrow have shown limited effectiveness in clinical trials (Sousa et al., 2014). The discovery of stem/progenitor cells in the brain and heart has raised hope for repairing damaged tissues caused by heart attacks and strokes (Mimeault & Batra, 2012).

As aforementioned, there are many potential sources of ASCs in the body, and the selection of isolation methods depends on the specific target. Bone marrow aspiration is a widely used technique for collecting ASCs from the bone marrow. It is a relatively safe procedure and provides a plentiful supply of stem cells. However, it can be invasive and may require the use of anaesthesia (Tomasian & Jennings, 2022). Umbilical cord blood banking offers a non-invasive approach to obtaining stem cells from the umbilical cord after childbirth. It carries no risk to the donor and provides a valuable source of stem cells. Nevertheless, the number of stem cells obtained may be limited, and challenges related to storage and availability may arise (Teofili et al., 2018). Adipose tissue, commonly known as fat, is another source of ASCs that can be accessed through liposuction. The advantage of this method lies within the yielded high number of stem cells. However, additional steps may be necessary to separate the stem cells from other cell types present in the tissue (Simonacci et al., 2019). Skin or muscle biopsies can also be utilised to obtain ASCs, but these methods are more invasive and carry the associated risks of a biopsy procedure (Kuci et al., 2009; Li et al., 2016).
Overall, the choice of isolation method depends on factors such as the desired quantity and quality of stem cells, invasiveness of the procedure, and availability of specialised equipment and expertise. As is the case with every laboratory and medical procedure, a thorough consideration of the advantages and disadvantages of each technique must be carefully conducted.
Conclusions
The field of stem cell research has made phenomenal progress in identifying various sources of stem cells and developing isolation methods for their extraction. The sources of stem cells include embryonic tissue, adult tissues, and genetically reprogrammed somatic cells. Each source offers unique advantages and considerations regarding availability, accessibility, and ethical considerations. Isolation methods range from simple and non-invasive techniques, such as umbilical cord blood banking, to more invasive procedures, like bone marrow aspiration or tissue biopsies. While ESCs, foetal stem cells, and iPSCs exhibit higher potency and can differentiate into numerous cell types, ASCs possess multipotent abilities limited to a specific range of cell types. Moreover, ASCs offer advantages such as overcoming ethical and legal concerns, avoiding mutational effects associated with iPSCs, and enabling autologous applications. In tissue engineering, ASCs can be combined with suitable scaffolds and influenced by various factors to achieve desired phenotypes. Among ASCs, adipose tissue stem cells hold promise due to their accessibility, non-invasiveness and abundant availability.
Bibliographic references
Abbott, A. (2013). Doubt cast over tiny stem cells. Nature, 499(7459), 390. https://doi.org/10.1038/499390a
Alstrup, T., Eijken, M., Bohn, A. B., Møller, B., & Damsgaard, T. E. (2019). Isolation of Adipose Tissue–Derived Stem Cells: Enzymatic Digestion in Combination with Mechanical Distortion to Increase Adipose Tissue–Derived Stem Cell Yield from Human Aspirated Fat. Current Protocols in Stem Cell Biology, 48(1), 1–13. https://doi.org/10.1002/cpsc.68
Bacakova, L., Zarubova, J., Travnickova, M., Musilkova, J., Pajorova, J., Slepicka, P., Kasalkova, N. S., Svorcik, V., Kolska, Z., Motarjemi, H., & Molitor, M. (2018). Stem cells: their source, potency and use in regenerative therapies with focus on adipose-derived stem cells – a review. Biotechnology Advances, 36(4), 1111–1126. https://doi.org/10.1016/j.biotechadv.2018.03.011
Bhartiya, D., Mohammad, S. A., Guha, A., Singh, P., Shama, D., & Kaushik, A. (2019). Evolving Definition of Adult Stem / Progenitor Cells. 1–3.
Bhartiya, D., Shaikh, A., Anand, S., Patel, H., Kapoor, S., Sriraman, K., Parte, S., & Unni, S. (2016). Endogenous, very small embryonic-like stem cells: Critical review, therapeutic potential and a look ahead. Human Reproduction Update, 23(1), 41–76. https://doi.org/10.1093/humupd/dmw030
Danova-Alt, R., Heider, A., Egger, D., Cross, M., & Alt, R. (2012). Very small embryonic-like stem cells purified from umbilical cord blood lack stem cell characteristics. PLoS ONE, 7(4), 1–11. https://doi.org/10.1371/journal.pone.0034899
English, K., & Wood, K. J. (2011). Immunogenicity of embryonic stem cell-derived progenitors after transplantation. Current Opinion in Organ Transplantation, 16(1), 90–95. https://doi.org/10.1097/MOT.0b013e3283424faa
Feng, B., Ng, J. H., Heng, J. C. D., & Ng, H. H. (2009). Molecules that Promote or Enhance Reprogramming of Somatic Cells to Induced Pluripotent Stem Cells. Cell Stem Cell, 4(4), 301–312. https://doi.org/10.1016/j.stem.2009.03.005
Gouveia, C., Huyser, C., Egli, D., & Pepper, M. S. (2020). Lessons learned from somatic cell nuclear transfer. International Journal of Molecular Sciences, 21(7). https://doi.org/10.3390/ijms21072314
Guenther, R., Dreschers, S., Maassen, J., Reibert, D., Skazik-Voogt, C., & Gutermuth, A. (2022). The Treasury of Wharton’s Jelly. Stem Cell Reviews and Reports, 18(5), 1627–1638. https://doi.org/10.1007/s12015-021-10217-8
Harris, J. (1997). “Goodbye dolly?“the ethics of human cloning. Journal of Medical Ethics, 353–360. https://doi.org/10.4324/9781315254517-19
Hosseini, V., Kalantary-Charvadeh, A., Hasegawa, K., Nazari Soltan Ahmad, S., Rahbarghazi, R., Mahdizadeh, A., Darabi, M., & Totonchi, M. (2020). A mechanical non-enzymatic method for isolation of mouse embryonic fibroblasts. Molecular Biology Reports, 47(11), 8881–8890. https://doi.org/10.1007/s11033-020-05940-3
Kuci, S., Kuci, Z., Latifi-Pupovci, H., Niethammer, D., Handgretinger, R., Schumm, M., Bruchelt, G., Bader, P., & Klingebiel, T. (2009). Adult Stem Cells as an Alternative Source of Multipotential (Pluripotential) Cells in Regenerative Medicine. Current Stem Cell Research & Therapy, 4(2), 107–117. https://doi.org/10.2174/157488809788167427
Landry, D. W., & Zucker, H. A. (2004). Embryonic death and the creation of human embryonic stem cells. Journal of Clinical Investigation, 114(9), 1184–1186. https://doi.org/10.1172/JCI23065
Li, Y., Nhuyen, H. V., & Tsang, S. H. (2016). Skin Biopsy and Patient-Specific Stem Cell Lines. Methods Mol Biol., 176(12), 77–88. https://doi.org/10.1007/7651
Liu, G., David, B. T., Trawczynski, M., & Fessler, R. G. (2020). Advances in Pluripotent Stem Cells: History, Mechanisms, Technologies, and Applications. Stem Cell Reviews and Reports, 16(1), 3–32. https://doi.org/10.1007/s12015-019-09935-x
Liu, S., Zhou, J., Zhang, X., Liu, Y., Chen, J., Hu, B., Song, J., & Zhang, Y. (2016). Strategies to optimize adult stem cell therapy for tissue regeneration. International Journal of Molecular Sciences, 17(6), 1–16. https://doi.org/10.3390/ijms17060982
Liu, Z., Cai, Y., Wang, Y., Nie, Y., Zhang, C., Xu, Y., Zhang, X., Lu, Y., Wang, Z., Poo, M., & Sun, Q. (2018). Cloning of Macaque Monkeys by Somatic Cell Nuclear Transfer. Cell, 172(4), 881-887.e7. https://doi.org/10.1016/j.cell.2018.01.020
Lukovic, D., Moreno-Manzano, V., Rodriguez-Jimenez, F. J., Vilches, A., Sykova, E., Jendelova, P., Stojkovic, M., & Erceg, S. (2017). HiPSC Disease Modeling of Rare Hereditary Cerebellar Ataxias: Opportunities and Future Challenges. Neuroscientist, 23(5), 554–566. https://doi.org/10.1177/1073858416672652
Mimeault, M., & Batra, S. K. (2012). Great promise of tissue-resident adult stem/progenitor cells in transplantation and cancer therapies. Advances in Experimental Medicine and Biology, 741, 171–186. https://doi.org/10.1007/978-1-4614-2098-9_12
Ofir, R. (2021). Hipsc-derived cells as models for drug discovery. International Journal of Molecular Sciences, 22(16), 2–5. https://doi.org/10.3390/ijms22168626
Pan, J., & Wan, J. (2020). Methodological comparison of FACS and MACS isolation of enriched microglia and astrocytes from mouse brain. Journal of Immunological Methods, 486(February), 112834. https://doi.org/10.1016/j.jim.2020.112834
Park, B., Yoo, K. H., & Kim, C. (2015). Hematopoietic stem cell expansion and generation: The ways to make a breakthrough. Blood Research, 50(4), 194–203. https://doi.org/10.5045/br.2015.50.4.194
Qin, Y., Qin, J., Zhou, C., Li, J., & Gao, W. Q. (2015). Generation of Embryonic Stem Cells from Mouse Adipose-tissue Derived Cells via Somatic Cell Nuclear Transfer. Cell Cycle, 14(8), 1282–1290. https://doi.org/10.1080/15384101.2015.1046331
Ratajczak, M. (2019). Stem cells : therapeutic applications.
Ratajczak, M. Z., Zuba-Surma, E., Kucia, M., Reca, R., Wojakowski, W., & Ratajczak, J. (2006). The pleiotropic effects of the SDF-1-CXCR4 axis in organogenesis, regeneration and tumorigenesis. Leukemia, 20(11), 1915–1924. https://doi.org/10.1038/sj.leu.2404357
Ratajczak, Mariusz Z., Zuba-Surma, E. K., MacHalinski, B., Ratajczak, J., & Kucia, M. (2008). Very Small Embryonic-Like (VSEL) Stem Cells: Purification from Adult Organs, Characterization, and Biological Significance. Stem Cell Reviews, 4(2), 89–99. https://doi.org/10.1007/s12015-008-9018-0
Shin, D. M., Liu, R., Klich, I., Ratajczak, J., Kucia, M., & Ratajczak, M. Z. (2010). Molecular characterization of isolated from murine adult tissues very small embryonic/epiblast like stem cells (VSELs). Molecules and Cells, 29(6), 533–538. https://doi.org/10.1007/s10059-010-0081-4
Simonacci, F., Bertozzi, N., Grieco, M. P., & Raposio, E. (2019). From liposuction to adipose-derived stem cells: Indications and technique. Acta Biomedica, 90(2), 197–208. https://doi.org/10.23750/abm.v90i2.6619
Sousa, B. R., Parreira, R. C., Fonseca, E. A., Amaya, M. J., Tonelli, F. M. P., Lacerda, S. M. S. N., Lalwani, P., Santos, A. K., Gomes, K. N., Ulrich, H., Kihara, A. H., & Resende, R. R. (2014). Human adult stem cells from diverse origins: An overview from multiparametric immunophenotyping to clinical applications. Cytometry Part A, 85(1), 43–77. https://doi.org/10.1002/cyto.a.22402
Storchová, Z. (2016). Too much to differentiate: aneuploidy promotes proliferation and teratoma formation in embryonic stem cells. 35(21), 2265–2267.
Sun, H., Zhang, F., Wang, Y., Wang, Z., Zhang, S., Xu, Y., & Shi, C. (2018). Generation of induced pluripotent stem cell line (ZZUi011-A) from urine sample of a normal human. Stem Cell Research, 29, 28–31. https://doi.org/10.1016/j.scr.2018.03.002
Suszynska, M., Zuba-Surma, E. K., Maj, M., Mierzejewska, K., Ratajczak, J., Kucia, M., & Ratajczak, M. Z. (2014). The proper criteria for identification and sorting of very small embryonic-like stem cells, and some nomenclature issues. Stem Cells and Development, 23(7), 702–713. https://doi.org/10.1089/scd.2013.0472
Takahashi, K., & Yamanaka, S. (2006). Induction of Pluripotent Stem Cells from Mouse Embryonic and Adult Fibroblast Cultures by Defined Factors. Cell, 126(4), 663–676. https://doi.org/10.1016/j.cell.2006.07.024
Teofili, L., Silini, A. R., Bianchi, M., Valentini, C. G., & Parolini, O. (2018). Incorporating placental tissue in cord blood banking for stem cell transplantation. Expert Review of Hematology, 11(8), 649–661. https://doi.org/10.1080/17474086.2018.1483717
Tomasian, A., & Jennings, J. W. (2022). Bone marrow aspiration and biopsy: techniques and practice implications. Skeletal Radiology, 51(1), 81–88. https://doi.org/10.1007/s00256-021-03882-w
Wilmut, I., Schnieke, A. E., McWhir, J., Kind, A. J., & Campbell, K. H. S. (1997). Viable offspring derived from fetal and adult mammalian cells. Nature, 385, 810–813.
Zhou, T., Benda, C., Dunzinger, S., Huang, Y., Ho, J. C., Yang, J., Wang, Y., Zhang, Y., Zhuang, Q., Li, Y., Bao, X., Tse, H. F., Grillari, J., Grillari-Voglauer, R., Pei, D., & Esteban, M. A. (2012). Generation of human induced pluripotent stem cells from urine samples. Nature Protocols, 7(12), 2080–2089. https://doi.org/10.1038/nprot.2012.115
Visual references
Cover Image [Image showing stem cells and parts of the body needing regeneration]. (2018). [Image]. Advanced Rejuvenation. https://www.advancedrejuvenation.us/other-services/vsel-stem-cells/
Figure 1. The two strategies for obtaining embryonic stem cells. (M. Ratajczak, 2019). [Illustration]. Stem cells : therapeutic applications.
Figure 2. Dolly: The Cloning of a Sheep. (n.d., 2023). [Image]. Encyclopædia Britannica. https://www.britannica.com/topic/Dolly-cloned-sheep
Figure 3. Cloned cynomolgus monkeys were generated by somatic cell nuclear transfer using fetal monkey fibroblasts. Liu, Z., Cai, Y., Wang, Y., Nie, Y., Zhang, C., Xu, Y., Zhang, X., Lu, Y., Wang, Z., Poo, M., & Sun, Q. (2018). [Illustration]. Cloning of Macaque Monkeys by Somatic Cell Nuclear Transfer. Cell, 172(4), 881-887.e7. https://doi.org/10.1016/j.cell.2018.01.020
Figure 4. Prof. Mariusz Ratajczak. (n.d.). [Photograph]. Foundation for Polish Science. https://www.fnp.org.pl/en/nagroda-dla-prof-mariusza-ratajczaka/
Figure 5. FACS and MACS techniques. Pan, J., & Wan, J. (2020). {Illustration]. Methodological comparison of FACS and MACS isolation of enriched microglia and astrocytes from mouse brain. Journal of Immunological Methods, 486(February), 112834. https://doi.org/10.1016/j.jim.2020.112834
Figure 6. Induced Pluripotent Stem Cells. (Louis A. Cona, 2023). DVCSTEM. https://www.dvcstem.com/post/adult-stem-cells
Figure 7. Induced Pluripotent Stem Cells in Regenerative Medicine. (n.d.). [Image]. Chemometec. https://chemometec.com/resources/industry/ipscs/
Figure 8. Pluripotent stem cells available for regenerative medicine. Bhartiya, D., Shaikh, A., Anand, S., Patel, H., Kapoor, S., Sriraman, K., Parte, S., & Unni, S. (2016). [Illustration]. Endogenous, very small embryonic-like stem cells: Critical review, therapeutic potential and a look ahead. Human Reproduction Update, 23(1), 41–76.
Figure 9. Comparison of Adult Stem Cells and Embryonic Stem Cells. (Louis A. Cona, 2023). DVCSTEM. https://www.dvcstem.com/post/adult-stem-cells